The Ocean
Chapter – 1
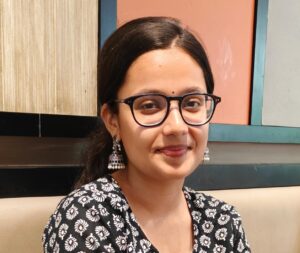
Table of Contents
- The ocean is a continuous body of salt water contained in enormous basins on Earth’s surface.
- Viewed from space, the oceans’ predominance on Earth is readily apparent.
- Oceans and their marginal seas cover nearly 71 percent of Earth’s surface, with an average depth of 3,795 metres (12,450 feet).
- Exposed land occupies the remaining 29 percent of the planetary surface and has a mean elevation of only 840 metres (2,756 feet).
- All the elevated land could be hidden under the oceans, reducing Earth to a smooth sphere completely covered by a continuous layer of seawater 2,686 metres (8,812 feet) deep, known as the sphere depth of the oceans.
- Earth is unique in the solar system due to its distance from the Sun and its period of rotation, subjecting it to a solar radiation level maintaining a mean surface temperature of 17 °C (62.6 °F).
- This mean temperature allows water to exist on Earth in all three phases—solid, liquid, and gaseous—unlike any other planet in the solar system.
- The liquid phase predominates on Earth, with 97.957 percent of the planet’s water existing as oceanic water and associated sea ice.
- The gaseous phase and droplet water in the atmosphere constitute 0.001 percent.
- Freshwater in lakes and streams makes up 0.036 percent, while groundwater is 10 times more abundant at 0.365 percent.
- Glaciers and ice caps constitute 1.641 percent of Earth’s total water volume.
- Each of these is considered a reservoir of water.
- Water continuously circulates between these reservoirs in the hydrologic cycle, driven by energy from the Sun.
- Evaporation, precipitation, movement of the atmosphere, and the downhill flow of river water, glaciers, and groundwater keep water in motion between the reservoirs and maintain the hydrologic cycle.
- The large range of volumes in these reservoirs and the rates at which water cycles between them create important conditions on Earth.
- Small changes in the rate at which water is cycled into or out of a reservoir can change the volume of that reservoir.
- Volume changes may be relatively large and rapid in a small reservoir or small and slow in a large reservoir.
- A small percentage change in the volume of the oceans may produce a large proportional change in the land-ice reservoir, promoting glacial and interglacial stages.
- The rate at which water enters or leaves a reservoir, divided by the reservoir volume, determines the residence time of water in the reservoir.
- The residence time of water in a reservoir governs many of the properties of that reservoir.
RELATIVE DISTRIBUTION OF THE OCEANS
- Oceanic research generally recognizes three major oceans: the Pacific, Atlantic, and Indian.
- The Arctic Ocean is considered an extension of the Atlantic.
- Arbitrary boundaries separate these three bodies of water in the Southern Hemisphere.
- One boundary extends southward to Antarctica from the Cape of Good Hope.
- Another boundary stretches southward from Cape Horn.
- The last boundary passes through Malaysia and Indonesia to Australia, and then on to Antarctica.
- Many subdivisions can be made to distinguish the limits of seas and gulfs with historical, political, and sometimes ecological significance.
- Water properties, ocean currents, and biological populations do not necessarily recognize these boundaries.
- Many researchers also do not adhere to these arbitrary boundaries.
- The oceanic area surrounding the Antarctic is considered by some to be the Southern Ocean.
- Boundaries must be established to separate individual regions for area-volume analyses of the oceans.
- In 1921, Erwin Kossina, a German geographer, published tables giving the distribution of oceanic water with depth for the oceans and adjacent seas.
- This work was updated in 1966 by H.W. Menard and S.M. Smith.
- Menard and Smith’s work only slightly changed the numbers derived by Kossina.
- Kossina’s original effort relied on sparse depth measurements accumulated by individual wire soundings.
- The more recent work had the benefit of acoustic depth soundings collected since the 1920s.
- This type of analysis, called hypsometry, allows quantification of the surface area distribution of the oceans and their marginal seas with depth.
- The distribution of oceanic surface area with 5° increments of latitude shows that land and water distribution on Earth’s surface is markedly different in the Northern and Southern hemispheres.
- The Southern Hemisphere may be called the water hemisphere, while the Northern Hemisphere is the land hemisphere.
- This is especially true in the temperate latitudes.
- The asymmetry of land and water distribution between the Northern and Southern hemispheres makes the two hemispheres behave very differently in response to the annual variation in solar radiation received by Earth.
- The Southern Hemisphere shows only a small change in surface temperature from summer to winter at temperate latitudes.
- This variation is controlled primarily by the ocean’s response to seasonal changes in heating and cooling.
- The Northern Hemisphere has one change in surface temperature controlled by its oceanic area and another controlled by its land area.
- In the temperate latitudes of the Northern Hemisphere, the land is much warmer than the oceanic area in summer and much colder in winter.
- This situation creates large-scale seasonal changes in atmospheric circulation and climate in the Northern Hemisphere that are not found in the Southern Hemisphere.
HYPSOMETRY
- Hypsometry is the science of measuring the elevation and depth of features on Earth’s surface with respect to sea level.
- Data collected using hypsometers, wire sounders, echo sounders, and satellite-based altimeters is used to quantify the distribution of land at different elevations across a given area.
- Hypsometry also quantifies the surface-area distribution of the oceans and their marginal seas with depth.
- Scientists can show how the areas of oceans, marginal seas, and terrestrial basins change with elevation and depth using a special curve known as a hypsometric, or hypsographic, curve.
MAJOR SUBDIVISIONS OF THE OCEANS
- If the volume of an ocean is divided by its surface area, the mean depth is obtained.
- The Pacific Ocean is the largest ocean in both surface area and volume, with or without marginal seas.
- The Atlantic Ocean is the second largest, followed by the Indian Ocean, which is the smallest.
- The Atlantic Ocean exhibits the largest change in surface area and volume when its marginal seas are subtracted, indicating it has the greatest area of bordering, shallow seas.
- Hypsometry can show how the area of each ocean or marginal sea changes as depth changes.
- A hypsometric, or hypsographic, curve portrays how the surface area of Earth is distributed with elevation and depth.
- This curve can represent the total Earth and all its oceans, or be constructed for each individual ocean and sea.
- The average depth of the world’s oceans is 3,795 meters (12,451 feet).
- The average elevation of the land is 840 meters (2,756 feet).
- The highest point on land is Mount Everest at 8,850 meters (29,035 feet).
- The deepest point in the ocean is located in the Mariana Trench at 11,034 meters (36,201 feet).
- The area under the hypsometric curve covering the 29.2 percent of Earth’s surface above sea level represents the volume of land above sea level.
- The area between sea level and the curve depicting the remaining 70.8 percent of Earth’s surface below sea level represents the volume of water contained in the oceans.
- Portions of the hypsometric curve describe the area of Earth’s surface that exists between elevation or depth increments.
- On land, only about 4 percent of Earth’s total area is at elevations above 2,000 meters (6,562 feet).
- Most of the land, 25.3 percent of the total Earth, is between 0 and 2,000 meters.
- About 13.6 percent of the total land area is at higher elevations, with 86.4 percent between 0 and 2,000 meters when the areas are determined relative to land area only.
- In the oceans, the percentages of the area devoted to depth increments yield information about the typical structure and shape of the oceanic basins.
- The small depth increment of 0–200 meters (0–660 feet) occupies about 5.4 percent of Earth’s total area or 7.6 percent of the oceans’ area.
- This depth approximates the world’s area of continental shelves, the shallow flat borderlands of the continents alternately covered by the oceans during interglacial stages and uncovered during glacial periods.
- At depths between 200 and 1,000 meters (about 660 and 3,300 feet) and between 1,000 and 2,000 meters (about 3,300 and 6,600 feet), an area only slightly larger—6.02 percent of Earth’s total area or 8.5 percent of the oceans’ area—is found.
- These depths are related to the regions of the oceans that have very steep slopes where depth increases rapidly, known as the continental slope regions marking the true edge of the continental landmasses.
- Marginal seas of moderate depths and the tops of seamounts add their area to these depth zones when all the oceans are considered.
- The majority of the oceanic area lies between 4,000 and 5,000 meters (about 13,100 and 16,400 feet).
- The continental shelf region varies immensely from place to place.
- The seaward boundary of the continental shelf historically is determined by the 100 fathom, or 200-meter, depth contour, though 85 fathoms, or 170 meters (about 560 feet), is a closer approximation.
- The true boundary at any given location is marked by a rapid change in slope of the seafloor known as the shelf break.
- This change in slope may be nearly at the coastline in areas where crustal plates converge, such as along the west coast of North and South America, or it may be located more than 1,000 km (620 miles) seaward of the coast, as off the north coast of Siberia.
- The average width of the shelf is about 75 km (47 miles), and the shelf has an average slope of about 0.01°, a slope that is barely discernible to the human eye.
- Seaward of the shelf break, the continental slope is inclined by about 4°.
ORIGIN OF OCEAN WATERS
- the huge volume of water contained in the oceans (and seas), 137 × 10^7 cubic km (about 33 × 10^7 cubic miles), has been produced during the geologic history of Earth.
- There is little information on the early history of Earth’s waters.
- Fossils dated from the Precambrian some 3.3 billion years ago show that bacteria and cyanobacteria (blue-green algae) existed, indicating the presence of water during this period.
- Carbonate sedimentary rocks, laid down in an aquatic environment, have been dated to 1 billion years ago.
- There is fossil evidence of primitive marine algae and invertebrates from the outset of the Cambrian Period some 542 million years ago.
- The presence of water on Earth at even earlier times is not documented by physical evidence.
- It has been suggested that the early hydrosphere formed in response to condensation from the early atmosphere.
- The ratios of certain elements on Earth indicate that the planet formed by the accumulation of cosmic dust and was slowly warmed by radioactive and compressional heating.
- This heating led to the gradual separation and migration of materials to form Earth’s core, mantle, and crust.
- The early atmosphere is thought to have been highly reducing and rich in gases, notably in hydrogen, and to include water vapor.
- Earth’s surface temperature and the partial pressures of the individual gases in the early atmosphere affected the atmosphere’s equilibration with the terrestrial surface.
- As time progressed and the planetary interior continued to warm, the composition of the gases escaping from within Earth gradually changed the properties of its atmosphere, producing a gaseous mixture rich in carbon dioxide (CO2), carbon monoxide (CO), and molecular nitrogen (N2).
- Photodissociation (separation due to the energy of light) of water vapor into molecular hydrogen (H2) and molecular oxygen (O2) in the upper atmosphere allowed the hydrogen to escape and led to a progressive increase of the partial pressure of oxygen at Earth’s surface.
- The reaction of this oxygen with the materials of the surface gradually caused the vapor pressure of water vapor to increase to a level at which liquid water could form.
- This water in liquid form accumulated in isolated depressions of Earth’s surface, forming the nascent oceans.
- The high carbon dioxide content of the atmosphere at this time would have allowed a buildup of dissolved carbon dioxide in the water and made these early oceans acidic and capable of dissolving surface rocks that would add to the water’s salt content.
- Water must have evaporated and condensed rapidly and accumulated slowly at first.
- The required buildup of atmospheric oxygen was slow because much of this gas was used to oxidize methane, ammonia, and exposed rocks high in iron.
- Gradually, the partial pressure of the oxygen gas in the atmosphere rose as photosynthesis by bacteria and photodissociation continued to supply oxygen.
- Biological processes involving algae increased, and they gradually decreased the carbon dioxide content and increased the oxygen content of the atmosphere until the oxygen produced by biological processes outweighed that produced by photodissociation.
- This, in turn, accelerated the formation of surface water and the development of the oceans.
COMPOSITION OF SEAWATER
- The chemical composition of seawater is influenced by a wide variety of chemical transport mechanisms.
- Rivers add dissolved and particulate chemicals to the oceanic margins.
- Wind-borne particulates are carried to mid-ocean regions thousands of kilometers from their continental source areas.
- Hydrothermal solutions that have circulated through crustal materials beneath the seafloor add both dissolved and particulate materials to the deep ocean.
- Organisms in the upper ocean convert dissolved materials to solids, which eventually settle to greater oceanic depths.
- Particulates in transit to the seafloor, as well as materials both on and within the seafloor, undergo chemical exchange with surrounding solutions.
- Through these local and regional chemical input and removal mechanisms, each element in the oceans tends to exhibit spatial and temporal concentration variations.
- Physical mixing in the oceans (thermohaline and wind-driven circulation) tends to homogenize the chemical composition of seawater.
- The opposing influences of physical mixing and of biogeochemical input and removal mechanisms result in a substantial variety of chemical distributions in the oceans.
DISSOLVED INORGANIC SUBSTANCES
- In contrast to the behavior of most oceanic substances, the concentrations of the principal inorganic constituents of the oceans are remarkably constant.
- Calculations indicate that, for the main constituents of seawater, the time required for thorough oceanic mixing is quite short compared with the time that would be required for input or removal processes to significantly change a constituent’s concentration.
- The concentrations of the principal constituents of the oceans vary primarily in response to a comparatively rapid exchange of water (precipitation and evaporation), with relative concentrations remaining nearly constant.
- Salinity is used by oceanographers as a measure of the total salt content of seawater.
- Practical salinity, symbol S, is determined through measurements of a ratio between the electrical conductivity of seawater and the electrical conductivity of a standard solution.
- Practical salinity can be used to calculate precisely the density of seawater samples.
- Because of the constant relative proportions of the principal constituents, salinity can also be used to directly calculate the concentrations of the major ions in seawater.
- The measure of practical salinity was originally developed to provide an approximate measure of the total mass of salt in 1 kg (2.2 pounds) of seawater.
- Seawater with S equal to 35 contains approximately 35 grams (1.2 ounces) of salt and 965 grams (34 ounces) of water.
- Inorganic phosphorus (HPO2−/4 and PO3−/4) and inorganic nitrogen (NO−/3, NO−/2, and NH+/4) are essential to the growth of marine organisms.
- Nitrogen and phosphorus are incorporated into the tissues of marine organisms in approximately a 16:1 ratio and are eventually returned to solution in approximately the same proportion.
- Dissolved inorganic phosphorus and nitrogen in oceanic waters exhibit a close covariance due to biological processes.
- Dissolved inorganic phosphorus distributions in the Pacific Ocean reflect phosphorus incorporation by organisms in surface waters and its return to solution via biological debris remineralized in the deep ocean.
- Inorganic phosphate concentrations in the western Pacific range from less than 0.1 micromole per kg at the surface to approximately 3 micromoles/kg at depth.
- Inorganic nitrogen ranges between less than 1 micromole/kg and 45 micromoles/kg along the same ocean section and exhibits covariance with phosphate.
- Elements essential to the growth of marine organisms, as well as some elements with no known biological function, exhibit nutrient-like behavior similar to nitrate and phosphate.
- Silicate is incorporated into the hard structural parts of certain marine organisms and exhibits a broad range of concentrations in oceanic waters.
- Zinc, essential to various biological functions, exhibits a distribution pattern generally parallel to that of silicate.
- Cadmium, though lacking a known biological function, generally exhibits distributions that are covariant with phosphate and concentrations lower than those of zinc.
- Essential trace metals like iron, cobalt, and copper show surface depletions but exhibit behavior more complex than phosphate, nitrate, and silicate.
- Adsorptive processes, either exclusive of or in addition to biological uptake, remove elements from the upper ocean and deliver them to greater depths, affecting their distribution patterns.
- Electron-exchange reactions lead to profound changes in the solubility and reactivity of trace metals in seawater, affecting the behavior of various elements including iron, manganese, copper, cobalt, chromium, and cerium.
- The processes delivering dissolved, particulate, and gaseous materials to the oceans ensure that nearly every element found in Earth’s crust and atmosphere is present in seawater, albeit at varying concentrations.
- The principal components of the atmosphere, such as nitrogen, oxygen, argon, and carbon dioxide, occur in seawater in variable proportions influenced by their solubilities and oceanic chemical reactions.
- Nitrogen and argon concentrations in seawater are influenced by equilibrium with the atmosphere, with their concentrations decreasing by approximately half at higher temperatures.
- The solubility behaviors of argon and oxygen are similar, with their concentrations in seawater having a ratio of approximately 20.45 when in equilibrium with the atmosphere.
- Oxygen concentrations in seawater exhibit variability due to biological processes, primarily exchange with the atmosphere, with minimum values often found at intermediate depths and higher values in deep waters.
- Estimates of oxygen utilization in the oceans can be obtained by comparing oxygen concentrations with those of argon, which is only influenced by physical processes.
- Dissolved oxygen concentrations generally mirror dissolved nutrient concentrations due to biological oxygen utilization and physical mixing of ocean waters.
- While the atmosphere holds a vast amount of oxygen compared to the oceans, the total carbon dioxide content of the oceans is significantly larger than that of the atmosphere.
- Carbon dioxide reacts with water in seawater to form carbonic acid, bicarbonate ions, and carbonate ions, with approximately 90% of total organic carbon present as bicarbonate ions.
- The formation of bicarbonate and carbonate ions from carbon dioxide releases hydrogen ions, which interact with various forms of inorganic carbon to buffer the acidity of seawater.
- The pH of seawater is maintained between 7.4 and 8.3 due to relatively high concentrations of total inorganic carbon and boron, which are essential for many reactions in seawater.
- Carbon dioxide produced by the combination of oxygen and organic carbon generally creates an acidity maximum (pH minimum) near the depth of the oxygen minimum in seawater.
- Dissolved inorganic carbon concentrations in seawater are also influenced by the formation and dissolution of calcareous shells of organisms abundant in the upper ocean.
DISSOLVED ORGANIC SUBSTANCES
- Processes involving dissolved and particulate organic carbon play a central role in shaping the chemical character of seawater.
- Marine organic carbon primarily originates in the uppermost 100 meters (330 feet) of the oceans, where dissolved inorganic carbon is converted to organic materials through photosynthesis.
- The “rain” of organic-rich particulate materials, resulting from photosynthetic production, directly and indirectly influences the distributions of many organic and inorganic substances in the oceans.
- A significant fraction of the vertical flux of materials in the uppermost waters is converted to dissolved substances within the upper 400 meters (about 1,300 feet) of the oceans.
- Dissolved organic carbon (DOC) constitutes at least 90 percent of the total organic carbon in the oceans, with surface ocean DOC concentrations ranging from roughly 100 to 500 micromoles of carbon per kg of seawater.
- DOC concentrations in the deep ocean are 5 to 10 times lower than surface values.
- DOC occurs in a wide variety of forms, and its composition is controversial and poorly understood, with conventional techniques identifying about 15 percent of DOC as carbohydrates and combined amino acids in surface waters.
- Approximately 1-2 percent of DOC in surface waters occurs as lipids, and 20-25 percent exists as relatively unreactive humic substances.
- The relative abundances of reactive organic substances, such as amino acids and carbohydrates, are considerably reduced in deep ocean waters.
- Dissolved and particulate organic carbon in the surface ocean undergoes diel cycles related to photosynthetic production and photochemical transformations.
- The influence of dissolved organic matter on ocean chemistry is often disproportionate to its oceanic abundance.
- Photochemical reactions involving DOC can impact the chemistry of vital trace nutrients such as iron, and even at dissolved concentrations as low as one nanomole/kg, dissolved organic substances in the upper ocean waters can greatly alter the bioavailability of essential trace nutrients, such as copper and zinc.
EFFECTS OF HUMAN ACTIVITIES
- Human activities have begun to influence the composition of the oceans on both a local and a global scale.
- Nutrient addition to coastal waters, such as through the discharge of untreated sewage or seepage of soluble mineral fertilizers, results in increased phytoplankton growth, high levels of dissolved and particulate organic materials, decreased light penetration through seawater, and alteration of the community structure of bottom-dwelling organisms.
- Industrial and automotive emissions have led to a dramatic increase in lead concentrations in the surface ocean on a global scale compared with preindustrial levels.
- Certain toxic organic compounds, like polychlorinated biphenyls (PCBs), are found in seawater and marine organisms and are solely attributable to human activities.
- While most radioactivity in seawater is natural, with about 90 percent being potassium-40 and less than 1 percent each as rubidium-87 and uranium-238, strontium-90 and certain other artificial radioisotopes have unique environmental pathways and potential for bioaccumulation.
- One of the most significant global-scale influences of human activities is the remarkable increase of carbon dioxide levels in the atmosphere.
- Atmospheric carbon dioxide levels are expected to double by the middle of the 21st century, with potentially profound consequences for global climate and agricultural patterns.
- The oceans, as a vast reservoir of carbon dioxide, are expected to mitigate this consequence of human activities to some degree.
THE PHYSICAL PROPERTIES OF SEAWATER
- Water is a unique substance, being the most abundant substance at Earth’s surface.
- It has the most naturally occurring physical states of any Earth material or substance: solid, liquid, and gas.
- Water has the greatest capacity to do things without being altered significantly.
- It is essential for sustaining life on Earth.
- Water affects the physical environment in myriad ways, such as sculpting landscape features through moving water, maintaining Earth’s radiation balance through atmospheric water vapor transfer, and transporting inorganic and organic materials around the planet’s surface via the oceans.
- The addition of salt to water changes the behavior of water only slightly.
SALINITY DISTRIBUTION
- The oceans are considered to be in steady state, receiving as much salt as they lose.
- The oceans have been mixed over such a long time period that the composition of sea salt is everywhere the same in the open ocean.
- This uniformity of salt content results in oceans where salinity varies little over space or time.
- Salinity in the open ocean ranges from 33 to 37 grams (1.2 to 1.3 ounces) of salt per kg of seawater or parts per thousand (0/00).
- Departures from the mean salinity value of approximately 35/00 are primarily caused by processes at Earth’s surface that locally add or remove fresh water.
- Regions of high evaporation have elevated surface salinities, while regions of high precipitation have depressed surface salinities.
- Nearshore regions close to large freshwater sources may have lowered salinity due to dilution, especially if the freshwater source is isolated from the open ocean by land geography.
- Areas of the Baltic Sea may have salinity values depressed to 100/00 or less.
- Increased salinity by evaporation is accentuated where isolation of the water occurs, such as in the Red Sea where surface salinity rises to 41/00.
- Coastal lagoon salinities in areas of high evaporation may be much higher.
- The addition or removal of fresh water by evaporation or precipitation does not affect the constancy of sea salt composition in the open sea.
- At depth in the oceans, salinity may be altered as seawater percolates into fissures associated with deep-ocean ridges and crustal rifts involving volcanism.
- Salt concentrations as high as 256/00 have been found in hot but dense pools of brine trapped in depressions at the bottom of the Red Sea, with compositions different from open ocean sea salt.
- Salinities of the open oceans found at greater depths are quite uniform in both time and space, with average values of 34.5 to 35/00, determined by surface processes.
- The intertropical convergence and subtropical zones influence surface salinity latitudinally, with high precipitation at the intertropical convergence and high evaporation at the subtropical zones causing variations in surface salinity.
HORSE LATITUDES
- The horse latitudes are two subtropical atmospheric high-pressure belts that encircle Earth around latitudes 30°–35° N and 30°–35° S.
- These belts generate light winds and clear skies due to the dry, subsiding air they contain.
- The areas below the horse latitudes often experience arid climates because of the dry conditions produced by the subsiding air.
- Examples of regions situated in horse latitudes include the Sahara Desert.
- The Southern Hemisphere has a more continuous belt of subsiding air in the horse latitudes due to its larger water area compared to the Northern Hemisphere.
- The horse latitudes contain several separate high-pressure centers and may shift a few degrees away from the equator in summer.
- The term “horse latitudes” originated from the practice of crews on sailing ships, who sometimes threw horses overboard to conserve water when their ships were becalmed in the high-pressure belts.
TEMPERATURE DISTRIBUTION
- Mid-ocean surface temperatures vary with latitude due to the balance between incoming solar radiation and outgoing long-wave radiation.
- Solar radiation excess occurs at latitudes less than approximately 45°, while radiation loss excess happens at latitudes higher than approximately 45°.
- Seasonal changes in solar radiation intensity and daylight duration, influenced by Earth’s axis tilt and rotation, further affect average ocean surface temperatures.
- Water’s high heat capacity results in downward heat mixing during summer surface heating and upward heat mixing during winter surface cooling, moderating the annual temperature cycle.
- In the tropics, ocean surface temperatures remain warm year-round, varying seasonally about 1 to 2°C (1.8 to 3.6°F). At mid-latitudes, surface temperatures vary about 8°C (14.4°F) over the year, while polar latitudes stay near the ice point of seawater.
- Land temperatures exhibit a large annual range at high latitudes due to the low heat capacity of the land surface.
- Prevailing winds carry cold air masses off continents in winter, cooling adjacent surface seawater, while warm continental air masses warm adjacent sea surfaces in summer, creating a greater annual temperature range at mid-latitudes.
- Ocean currents transport water with specific characteristics between latitudinal zones, creating sharp temperature changes along current boundaries and thermal fronts.
- Trade winds at low latitudes move water away from lee coasts, producing areas of coastal upwelling and reducing surface temperatures.
- Ocean temperatures decrease with depth, with no seasonal changes at greater depths. Temperature at depth is determined by conditions encountered when water was last at the surface.
- The formation of dense water at the surface in high temperate and Arctic regions produces nearly isothermal conditions with depth.
- Areas experiencing an annual change in surface heating develop a seasonal thermocline below a shallow wind-mixed layer in summer, while convective overturning erases the shallow thermocline and deepens the isothermal layer in winter.
- Below the permanent thermocline, temperatures decrease slowly, but in very deep ocean basins, temperatures may slightly increase with depth due to adiabatic temperature rise caused by compression.
- Adiabatic temperature rise is calculated and subtracted from observed deep-sea temperatures to obtain potential temperatures, which are used to identify water types and trace them back to their source.
THERMAL PROPERTIES
- The gram calorie is the amount of heat required to raise the temperature of 1 gram of water by 1°C, while the kilocalorie is the amount of heat needed to raise 1 kg of water by 1°C.
- Water’s heat capacity is 1 kilocalorie per kg per degree Celsius in the International System of Units (SI), making it the highest among common Earth materials.
- Heat capacity divided by the heat capacity of water yields the specific heat of a material, which is numerically equal to heat capacity but without units.
- The specific heat of seawater decreases slightly in the presence of salt, with seawater at 35‰ having a specific heat of 0.932 compared to 1.000 for pure water.
- The addition of salt alters the freezing and boiling points of water, with the freezing point lowered and the boiling point raised. Salt also lowers the temperature of maximum density of water below that of pure water.
- The temperature of maximum density decreases faster than the freezing point as salt concentration increases, leading to scenarios where maximum density is never reached before freezing occurs.
- Water undergoes changes in state by forming or breaking hydrogen bonds between molecules, requiring energy input to break bonds and releasing energy when bonds are formed.
- The latent heat of fusion, required to change water from solid to liquid, is 80 calories per gram of ice, the highest among common materials.
- Water’s latent heat of vaporization, needed to convert liquid water to vapor, is 540 calories per gram at 100°C. Evaporation below the boiling point and sublimation require more energy per gram than 540 calories.
- When water vapor condenses back to liquid, the latent heat of vaporization is released, contributing to processes like hurricane formation and ocean cooling.
- The latent heat of vaporization of water is the highest among common substances, making evaporation a crucial mechanism for transferring heat from Earth’s surface to the atmosphere.
DENSITY OF SEAWATER AND PRESSURE
- Density is expressed in units of mass per unit volume, typically kilograms per cubic meter in the SI system.
- In oceanography, seawater density has historically been expressed in grams per cubic centimeter.
- Seawater density is influenced by temperature, salinity, and pressure.
- Accuracy in density measurements, often required to the fifth decimal place, necessitates recording many numbers for each measurement.
- Pressure effects can sometimes be neglected by using potential temperature.
- To simplify density representation, oceanographers use a unit called sigma-t (σt), obtained by subtracting 1.0 from the density and multiplying the remainder by 1,000.
- Sigma-t has no units and serves as an abbreviated density value controlled by salinity and temperature.
- The sigma-t of seawater increases with increasing salinity and decreasing temperature.
- Pressure and density are related, as demonstrated by observing the effect of pressure on seawater density at 35‰ and 0°C (32°F).
- A 1-meter column of seawater produces a pressure of about 1 decibar, approximately equal to the depth in meters.
- One decibar is one-tenth of a bar, equivalent to 10^5 newtons per square meter.
- Increasing density values illustrate the compressibility of seawater under the immense pressures in the deep ocean.
- Calculations show that the average pressure over 4,000 meters (13,100 feet), the approximate mean depth of the ocean, is approximated by that at 2,000 meters (about 6,600 feet).
- The average volume change due to pressure for each gram of water in the entire water column is calculated to be about 0.00876 cm^3/g.
- Considering the number of grams of water in a column of seawater 4 × 10^5 cm in length, and the average density of the water, the expansion of the entire water column results in an average sea level rise of about 36 meters (118 feet) if the area of the oceans is considered constant.
- The temperature of maximum density and the freezing point of water decrease as salt is added, with the temperature of maximum density decreasing more rapidly than the freezing point.
- At salinities less than 24.70‰, the density maximum is reached before the ice point, while at higher salinities more typical of the open oceans, the maximum density is never naturally achieved.
- Low-salinity water and fresh water behave differently from marine systems during surface cooling and density-driven overturn due to their ability to pass through a density maximum.
- In lakes, during fall, surface water sinks as it cools until it reaches 4°C, the temperature of maximum density for fresh water, leading to stable stratification with respect to temperature-controlled density.
- In deep lakes, the temperature at depth remains at 4°C, and in spring, surface water warms up and ice melts, with a shallow convective overturn resuming until the lake is once more isothermal at 4°C.
- In seawater with salinity exceeding 24.70‰, convective overturn occurs during the cooling cycle until the ice point is reached, forming sea ice and increasing the salinity of the water beneath the ice.
- The continuing overturn results in the formation of very dense seawater, known as Antarctic Bottom Water, which sinks to the deepest depths of the oceans, limiting the thickness of seasonal sea ice.
- Other factors that control the thickness of ice include the rate of heat conduction through the ice layer and the insulation provided by snow on the ice, with seasonal sea ice seldom exceeding about 2 meters in thickness.
- During warmer seasons, melting sea ice supplies a freshwater layer to the sea surface, stabilizing the water column.
- Surface processes altering the temperature and salinity of seawater drive vertical circulation, known as thermohaline circulation, continually replacing seawater at depth with surface water and vice versa.
OPTICAL PROPERTIES
- Water is transparent to the wavelengths of electromagnetic radiation within the visible spectrum but opaque to wavelengths above and below this band.
- Visible light entering water at angles other than a right angle is refracted due to the slower speed of light waves in water compared to air, with the refractive index affected by salinity and temperature.
- The refractive index of seawater at a constant temperature can be used to determine the salinity of the sample.
- Some of the Sun’s radiant energy is reflected at the ocean surface, while the energy that penetrates is attenuated by absorption, conversion to other forms of energy, or scattering by molecules and suspended particulates.
- Upward scattered light and light reflected from particles determine the color of the oceans as seen from above.
- Water molecules, dissolved salts, organic substances, and suspended particulates combine to decrease the intensity of solar radiation with depth, with different wavelengths being attenuated at different rates.
- Blue-green wavelengths penetrate the deepest and are most available for scattering, giving the oceans their blue appearance.
- Changes in ocean water color can be caused by the color of suspended particulates and dissolved substances or by variations in solar radiation quality at the ocean surface due to the angle of the Sun and atmospheric conditions.
- Light attenuation in oceans can be measured using methods like the Secchi disk, which yields an attenuation coefficient for available light averaged over its depth. Beer’s law can be applied to estimate light intensity at depth using this coefficient.
- The Secchi disk method does not indicate the attenuation change with depth or the attenuation of specific wavelengths of light.
- Photocells can be lowered into the ocean to measure light intensity at discrete depths and determine light reduction from surface values or previous depth values.
- They may sense all available wavelengths or be equipped with filters passing only certain wavelengths of light.
- By knowing Iz and I0, changing light intensity values can be used in Beer’s law to determine how the attenuation coefficient changes with depth and the quality of light.
- These measurements help determine the level of photosynthesis as a function of radiant energy level with depth and measure changes in water turbidity caused by particulate distribution.
- Different ocean areas have different optical properties; near rivers, silt increases suspended particle effect, while nutrient-rich areas with abundant sunlight may have increased opacity due to phytoplankton.
- Solar radiation received at the ocean surface varies in time and space due to factors like cloud cover, atmospheric dust, atmospheric gas composition, ocean surface roughness, and the elevation angle of the Sun.
- Reflectance is low when the Sun’s rays are perpendicular to a smooth ocean surface and increases when the rays are oblique or when the ocean is rough with waves.
- Light penetration is generally less at higher latitudes due to the Sun’s lower elevation angle.
- Factors like cloud cover, density layering, fog, and dust cause refraction and atmospheric scattering of sunlight, affecting its penetration into the ocean.
- Strongly scattered sunlight results in non-unidirectional rays, eliminating shadows and allowing light to enter the ocean from all angles.
- The solar energy available to penetrate the ocean is 100 percent minus the reflectance value.
ACOUSTIC PROPERTIES
- Water is an excellent conductor of sound, better than air, and sound attenuation depends on frequency and water properties.
- The attenuation coefficient in Beer’s law for sound is dependent on water viscosity, inversely proportional to sound frequency and water density.
- Sound velocity in water is determined by the square root of elasticity divided by water density, with changes in temperature, salinity, and pressure affecting sound velocity.
- In the oceans, sound speed varies between 1,450 and 1,570 meters per second, increasing with temperature, salinity, and pressure.
- Changes in salinity and temperature near the surface have the greatest effect on sound speed, while pressure becomes more important deeper in the ocean.
- Sound speed changes caused by variations in salinity and temperature can result in sound wave refraction, affecting sonar detection and producing shadow zones.
- At depths of around 1,000 meters, pressure, temperature, and salinity combine to create a zone of minimum sound speed called the SOFAR channel, where sound waves can travel long distances.
- Marine animals rely on hearing for communication and object detection, using directional sound signals and echoes to locate targets and gain information about their properties.
CHEMICAL EVOLUTION OF THE OCEANS
- The chemical history of the oceans is divided into three stages.
- The first stage occurred during the early formation of the Earth’s crust, characterized by cooling and reactions with volatile or highly reactive gases, leading to the formation of the oceans and initial sedimentary rock mass. This stage lasted until about 3.5 billion years ago.
- The second stage marked a transition towards essentially modern conditions and is estimated to have ended 2 to 1.5 billion years ago.
- Since the end of the second stage, it is likely that there has been little change in seawater composition.
THE EARLY OCEANS
- The initial accretion of Earth occurred around 4.6 billion years ago through the agglomeration of solid particles.
- Heating of the initially cool conglomerate was caused by the decay of radioactive elements and the conversion of kinetic and potential energy to heat.
- This heating resulted in the development of a liquid iron core and the gross internal zonation of Earth.
- It’s estimated that the formation of Earth’s core took about 500 million years.
- Core formation likely led to the escape of an original primitive atmosphere and its replacement by one derived from the loss of volatile substances from Earth’s interior.
- Whether most of this degassing occurred during core formation or soon afterward, or if there has been significant degassing throughout geologic time, is uncertain.
- Recent models of Earth formation suggest early differentiation into three major zones (core, mantle, and crust) and early loss of volatile substances from the interior.
- After initial cold agglomeration, Earth likely reached temperatures where the entire planet approached the molten state.
- As the initial crust solidified, volatile gases would have been released to form an atmosphere containing water, carbon gases (such as carbon dioxide, methane, and carbon monoxide), sulfur gases (mostly hydrogen sulfide), and halogen compounds (like hydrochloric acid).
- Nitrogen may also have been present, along with minor amounts of other gases.
- Gases of low atomic number, such as hydrogen and helium, would have escaped Earth’s gravitational field.
- Substances degassed from the planetary interior have been termed excess volatiles because their masses cannot be accounted for simply by rock weathering.
- At an initial crustal temperature of about 600°C (1,100°F), almost all these compounds, including water (H2O), would have been in the atmosphere.
- Below 100°C (212°F), all the water would have condensed, and the acid gases would have reacted with the original igneous crustal minerals to form sediments and an initial ocean.
- There are at least two possible pathways by which these initial steps could have been accomplished.
- One pathway suggests that the 600°C (1,100°F) atmosphere contained water vapor, carbon dioxide, and hydrochloric acid in a specific ratio, which would cool to the critical temperature of water, leading to the condensation of water vapor into an early hot ocean.
- In this scenario, hydrochloric acid would dissolve in the ocean, while most of the carbon dioxide would still be in the atmosphere.
- This early acidic ocean would react vigorously with crustal minerals, dissolving silica and cations, creating a residue consisting mainly of aluminous clay minerals that would form the sediments of the early ocean basins.
- Another pathway assumes slower cooling, where most of the water vapor would be removed from the atmosphere by hydration reactions with pyroxenes and olivines around 400°C (750°F).
- Under these conditions, water vapor wouldn’t condense until reaching some unknown temperature, potentially resulting in an atmosphere rich in carbon dioxide and no ocean, resembling present-day Venus.
- Regardless of the pathway, once Earth’s surface cooled to 100°C (212°F), it would take a short geological time for acid gases to be used up in reactions involving igneous rock minerals.
- Evidence from fossils older than 3 billion years suggests that Earth’s surface had cooled by this time, and neutralization of the original acid gases had taken place.
- If most degassing occurred early, chloride released by reaction of hydrochloric acid with rock minerals would be found in the oceans, leading to a salinity and volume comparable to today.
- The conclusion is based on the assumption of no drastic change in volatile release ratios over geologic time.
- The overall generalized reaction leading to the formation of early oceans can be written as: primary igneous rock minerals + acid volatiles + H2O → sedimentary rocks + oceans + atmosphere.
- The salt content of the oceans, based on constant proportions of released volatiles, primarily depends on the ratio of sodium chloride (NaCl) locked up in evaporites to that dissolved in the oceans.
- Early terrestrial surfaces lacked free oxygen, which wasn’t a constituent released from the cooling crust.
- Oxygen production began through photodissociation of water in the atmosphere due to absorption of ultraviolet light, followed by reactions with early reduced gases.
- However, it wasn’t until the appearance of photosynthetic organisms around 3.3 billion years ago that oxygen accumulation in the atmosphere could proceed at a rate leading to today’s oxygenated environment.
THE TRANSITION STAGE
- The rock record from about 3.5 billion years ago to 2 to 1.5 billion years ago suggests lower oxygen levels in the atmosphere and continuous chemical trends in sedimentary rocks and oceanic composition.
- Sedimentary detritus during this time likely formed from the alteration of more basaltic source rocks in an oxygen-deficient atmosphere, accumulating primarily under anaerobic marine conditions.
- During this period, oxygen-deficient environments favored the formation of minerals containing ferrous iron from the alteration of basaltic rocks.
- Minerals like iron carbonate siderite, iron silicate greenalite, chert, and iron sulfide pyrite are characteristic of middle Precambrian iron formations.
- The oceans at this time resulted from an acid leach of basaltic rocks, likely occurring through submarine processes due to an oxygen-deficient atmosphere.
- Early ocean water composition likely had a lower pH, higher calcium concentration, and was saturated with respect to amorphous silica.
- The simulation of early ocean formation involves neutralizing hydrochloric acid leaching basaltic rocks, leading to the production of a chloride ocean with specific mineral compositions.
- Sediments produced in this system would contain silica, ferrous iron silicates, chloritic minerals, calcium carbonate, calcium magnesium carbonates, and minor pyrite.
- Excess hydrochloric acid would result in a high content of calcium chloride in the ocean, while excess carbon dioxide would precipitate calcium carbonate until reaching levels similar to present oceans.
- Over time, these newly formed oceans would interact with continental debris, potentially shifting pH slightly and oxidizing iron out of ferrous silicates to produce iron oxides.
- The primary minerals of igneous rocks, reacting with acids like hydrochloric acid and carbon dioxide, produce neutral or mildly alkaline solutions plus altered aluminosilicate and carbonate reaction products.
- Ocean water composition likely remained in equilibrium with these reaction products, including clay minerals and carbonates, throughout geological time.
THE MODERN OCEANS
- The oceans likely attained their modern characteristics 2 to 1.5 billion years ago, with little difference in chemical and mineralogical compositions compared to Paleozoic counterparts.
- Late Precambrian deposits, approximately 570 million to 1.6 billion years old, show evidence of neutralized acid sulfur gases and the presence of available free oxygen indicated by chemically precipitated ferric oxides.
- Middle and late Precambrian shales exhibit similar chemistry and mineralogy to Paleozoic shales, suggesting continuous cycling of sediments for 1.5 to 2 billion years and their influence on oceanic composition.
- Initially, it was believed that the saltiness of modern oceans resulted from salts derived from rock weathering and transported by fluvial processes. However, considering Earth’s age, it became apparent that this accumulation could occur rapidly compared to geological time scales.
- The oceans function as a steady-state system, where rates of influx of materials into the oceans are balanced by rates of outflow, allowing for variations in influx to be matched by nearly simultaneous variations in efflux.
- Calculations indicate that for at least 100 million years, the oceanic system has maintained a steady state with fixed rates of major element inflow and outflow, leading to a stable chemical composition.